Resistance fighters
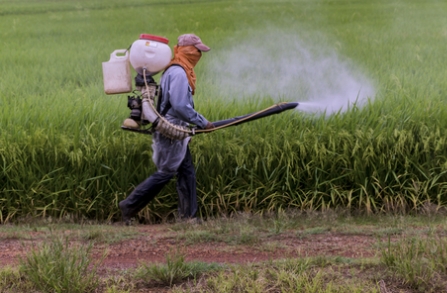
Just like bacteria, agricultural pests evolve resistance to the chemicals we develop to control them. Anthony Flemming looks at what we can do about it
The Biologist Vol 63(6) p16-19
For an increasingly urbanised population, the agricultural landscape is a distant view from the car on long journeys – often beautiful and peaceful, but far removed from our daily lives. Look closely, though, and the fields are battlefields: human versus pest. Agricultural pests include herbivorous insects, weeds and crop pathogens, and collectively they take a big bite out of crop yields worldwide – all forms of agriculture try to control them[1]. But the pests fight back.
Resistance sounds familiar in the context of healthcare – antibiotic resistance and drug-resistant cancer are both in the public consciousness to a degree. Far less familiar is resistance in agriculture, and yet the scale of it is staggering. The International Survey of Herbicide Resistant Weeds has recorded resistance to 160 herbicides in 250 weed species affecting 86 crops in 66 countries[2]. And it is not just herbicides – resistant insects and fungi are widespread, too. All major types of insecticides, herbicides and fungicides have significant resistance problems somewhere in the world.
Most pesticides are synthetic, organic molecules that, when introduced to a pest, chemically disrupt the biology of the organism, leading to its death. Typically, the molecule specifically binds to a single 'target' protein that is critical to the pest's survival. Once bound, the function of the protein is altered to a greater extent than the pest can tolerate and it dies.
Survival tactics
Resistance evolves through changes that affect either the access of the pesticide to the target protein or the target protein itself. In other words, resistant strains may stop the pesticide getting to its target protein or have an altered target protein that has reduced sensitivity to the pesticide. Some resistant strains combine both tactics.
Probably the best-studied mechanism by which a pesticide is prevented from reaching its target protein is metabolism. All organisms have enzymes that break down chemicals, including pesticides.
Once broken down, the pesticide will no longer work. If this process works well, not enough of the pesticide will reach its target protein to have an effect. Because of this, some resistant species have greatly enhanced their metabolic capabilities. For example, a strain of whitefly (Bemisia tabaci) that has evolved resistance to neonicotinoid pesticides has increased the expression of a metabolic enzyme called CYP6CM1. With more of this metabolic enzyme, they can break down the neonicotinoid better and survive[3].
Along a similar principle, some resistant strains are better at excreting or storing pesticides away from their target proteins. Plant cells contain large vacuoles, which are, in part, dumping grounds for unwanted chemicals produced by the plant, or to which the plant has been exposed.
Horseweed (Conyza canadensis) is an arable weed, originally from North America but now found worldwide. It has developed resistance to glyphosate (which inhibits the enzyme 5-enolpyruvylshikimate-3-phosphate synthase) by more effectively pumping it into the vacuole and therefore away from the target enzyme[4].
Just as effective at delivering resistance, though, are changes in the target protein itself. Sometimes resistant strains simply make more of the target protein – more than the standard dose of pesticide can affect – which then leads to resistance.
Pesticides are designed to be a perfect fit to a binding site on their target protein. Small changes in the binding site can prevent a pesticide from working properly – the substitution of a single amino acid with another is often enough (see Fig. 1). Grey mould (Botrytis cinerea) – a plant pathogenic fungus – has evolved resistance to the fungicides carbendazim (target protein: tubulin), iprodione (target: histidine kinase), strobilurin (target: cytochrome bc1), fenhexamid (target: 3-keto-reductase) and boscalid (target: succinate dehydrogenase) by substituting one amino acid for another in the respective target protein[5].
One of the best-studied target proteins in insects is the voltage-gated sodium channel. These channels are important components of the insect nervous system and are targeted by modern pyrethroid insecticides, as well as by older, now defunct, insecticides such as DDT. Resistance was first observed in the 1950s in the housefly (Musca domestica), but has now evolved many times in agricultural, animal and human pests.
More than 30 mutations in the gene encoding the voltage-gated sodium channel in insects are associated with resistance[6]. The most common is the substitution of the amino acid leucine with phenylalanine at position 1014 (that is, the 1014th amino acid along the length of the protein). This is known as the knock-down resistant (kdr) mutation, as the insects are no longer 'knocked down' by the insecticide.
Some 20 insect species have evolved resistant strains bearing this mutation, and several more have a similar mutation where an amino acid other than phenylalanine or leucine is found at the same position. Mutations at this position alone confer a five- to tenfold reduction in sensitivity of the channel to deltamethrin (a pyrethroid insecticide) in the lab. This is a problem in itself, but when this mutation co-occurs with a second mutation in the same gene (methionine substituted by leucine at position 918, termed 'super kdr'), the channel becomes virtually insensitive to deltamethrin. Several insect species have evolved resistant strains with this double mutation.
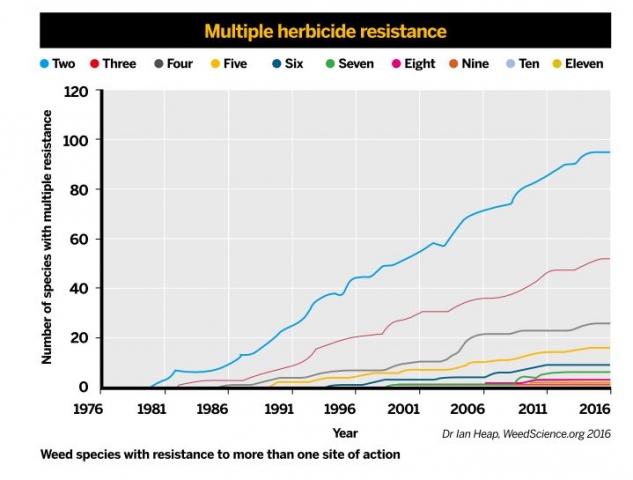
So resistance to any given pesticide can result from a number of mechanisms either acting in isolation or in concert – some resistant insects and weeds seem to combine target site and metabolic mechanisms, for example. There are other mechanisms of resistance that are rather less well studied and may also be important components of resistance. Some resistant insects apparently have changes in their cuticle, which limits entry of the insecticide. Moreover, there are reports of resistance where strains alter behaviour, such as where they lay their eggs to minimise contact with insecticides when they are applied.
Of particular worry are strains that combine multiple resistance mechanisms to multiple pesticides. A strain of annual ryegrass (Lolium rigidum), an arable weed, combines four mechanisms of resistance to three herbicides[7]. First, it is resistant to glyphosate via a target site mutation and by reduced translocation of the herbicide through the plant. Second, it is resistant to paraquat, also by restricting movement of the herbicide, but likely by a different mechanism to the reduced movement of glyphosate. Third, it is resistant to several herbicides inhibiting acetyl CoA carboxylase (ACCase), such as tralkoxydim, through an amino acid substitution in the target protein.
An especially troublesome weed in the UK is black-grass (Alopecurus myosuroides), which has evolved metabolic and target-site resistance to many herbicides, making it very difficult to control[8]. Similarly, there are Asian strains of the diamondback moth (Plutella xylostella) – a pest of cruciferous vegetables worldwide – that appear to have target site resistance as well as metabolism-based resistance to insecticides acting at several different target proteins.
Survival of the fittest
Darwin was fascinated by agriculture, and learned much about natural selection from studying the artificial selection that had produced the diverse breeds and cultivars used in agriculture. Indeed, his most substantial work is The Variation of Animals and Plants under Domestication[9]. Resistance is a demonstration of his theory in action on a global scale. The evolution of resistant strains happens, of course, because the genetic traits that cause resistance enable individuals to survive and reproduce, passing on those traits to the next generation, and so on. The resistant traits, therefore, become more common within the pest population to the point where they are so abundant that growers find their crop protection chemistry loses efficacy.
However, there is considerable complexity underlying this. Genetic mutations naturally occur in all organisms and result in genetic variability among individuals within wild populations. Where this variability affects the individual's resistance to a pesticide, this will become the basis of resistance evolution. Some resistance mutations seem to be harmful to the organisms' fitness, so in the absence of selection by a pesticide, these will be selected against and hence be rare or only sporadically present in the wild.
The fitness cost, and the consequent abundance of a resistance mutation when a pesticide is first introduced, likely contribute to the rate of resistance evolution to that pesticide.
Furthermore, resistance can be what geneticists call a complex trait – in other words, a combined effect of mutations (sometimes more than one) in several genes. The rate at which a complex resistance genotype will assemble in individuals of a population under selection, and how quickly this genotype will sweep through the population, is hard to predict and will depend on many factors. These include the selection pressure itself (area, frequency, uniformity, dosage, efficacy, duration, etc, of pesticide application); the properties of the species (distribution, fecundity, generation time, reproductive mode, etc); and the genes (constraint, allelic diversity, heterozygosity, etc) under selection.
All these factors will vary considerably, which means understanding the progression and mechanisms of resistance evolution for particular pests and pesticides remains a central challenge in resistance research. It is often remarked that some pests evolve resistance more easily than others – the Colorado potato beetle (Leptinotarsa decemlineata), for example (see 'I will survive', below) – but why this is so remains unclear.
What can growers and the crop protection industry do to combat resistance? Resistance mechanisms are often specific to particular types of chemistry and particular target proteins so, as for antibiotics, the invention of new chemistries acting against novel target proteins is an obvious solution.
We can also work to best preserve the efficacy of the pesticides already in use. A scientific challenge is to identify resistance mechanisms as they evolve; a practical challenge is to detect and monitor the incidence and distribution of these mechanisms worldwide. With cheaper DNA sequencing costs, it is possible to directly determine the presence of a resistance mutation in the pest and the ongoing development of portable sequencing technology will only make this easier.
Resistance is futile
It is also possible to design simple diagnostic tests for particular resistance mechanisms. For example, the Resistance In-Season Quick (RISQ) test developed by Syngenta allows seedlings collected on farm to be rapidly assessed for resistance to two major classes of herbicide – those targeting acetolactate synthase and ACCase. The appropriate response can then be made based on the result[10].
The response may be to use an alternative pesticide to which resistance is not present. This enables the farmer to control the pest, but also destroys the genotypes present on the farm that are resistant to other pesticides and so prevent their spread. In general, rotation of pesticides with differing target proteins (differing 'mode of action' in the language of the industry), is an important defence against resistance evolution by preventing sustained selection for any specific resistance mechanism.
To support this, the crop-protection chemistry industry has formed the Insecticide, Fungicide and Herbicide Resistance Action Committees (IRAC, FRAC and HRAC respectively – links below). These provide a unified industry-wide classification of pesticides by mode of action, enabling growers to identify alternative pesticides to rotate and minimise resistance selection. They also act as information hubs, collating new research on resistance worldwide and communicating expert recommendations for resistance management.
Alongside better use of pesticides, the principles of integrated pest management are likely a useful tool for resistance management. Here, growers are, among other things, advised to use multiple tactics in the control of pests, which might include crop rotation, biological control agents, encouragement of the pests' natural enemies, crop-protection chemistry and so forth. Any diversification of methods used to control pests will likely mitigate resistance evolution, as it will avoid selection for any single resistance mechanism[11].
Although much is known and much can be done about resistance, it remains a challenge. Our knowledge of resistance mechanisms is patchy and our understanding of the evolutionary processes leading to resistance is very limited. If we could combine understanding of these two areas – mechanism and evolution – we might better predict the progress of resistance evolution and counter it before it saturates pest populations[12]. Expanding the scientific study of resistance, be it in industry, academia or elsewhere, and translation of this to agricultural practice is something we would all benefit from. Perhaps we can hope that, under these circumstances, our management of pest resistance will be far from futile.
I will survive: The Colorado potato beetle
The Colorado potato beetle (Leptinotarsa decemlineata) is found only sporadically in the UK, but any sightings must be reported to DEFRA. This rapacious herbivore of commercially grown potatoes has a legendary ability to evolve resistance to pesticides.
While not all populations have the same resistance abilities, the Arthropod Resistance Database records that the species as a whole has evolved resistance to 56 chemical insecticides. There is even a report from Ukraine in the 1970s of a strain resistant to hydrogen cyanide.
It is said that during the Cold War, the Soviet Union suspected that the beetle was part of a CIA plot to threaten food security. The mechanisms of resistances include several metabolic enzymes and target site mutations, reduced penetration and increased excretion of insecticide, as well as evolved avoidance behaviour.
1) Oerke, E. C. Crop losses to pests. Journal of Agricultural Science 144(1), 31–43 (2005).
2) Heap, I. The International Survey of Herbicide Resistant Weeds www.weedscience.com
3) Karunker, I. et al. Structural model and functional characterization of the Bemisia tabaci CYP6CM1vQ, a cytochrome P450 associated with high levels of imidacloprid resistance. Insect Biochem. and Molecular Bio. 39(10), 697–706 (2009).
4) Ge, X. et al. Rapid vacuolar sequestration: the horseweed glyphosate resistance mechanism. Pest Management Science 66(4), 345–348 (2010).
5) Hahn, M. The rising threat of fungicide resistance in plant pathogenic fungi: Botrytis as a case study. Journal of Chemical Biology 7(4), 133–141 (2014).
6) Rinkevich, F. D. et al. Diversity and convergence of sodium channel mutations involved in resistance to pyrethroids. Pesticide Biochemistry and Physiology 106(3), 93–100 (2013).
7) Yu, Q. et al. Glyphosate, paraquat and ACCase multiple herbicide resistance evolved in a Lolium rigidum biotype. Planta 225(2) 499–513 (2007).
8) Moss, S. R. et al. Managing herbicide-resistant black-grass (Alopecurus myosuroides): Theory and Practice. Weed Technology 21(2), 300–309 (2007).
9) Darwin, C. The Variation of Animals and Plants under Domestication. Vol. 1 & 2. (John Murray, London, 1868).
10) Kaundun, S. S. et al. Syngenta 'RISQ' test: a novel in-season method for detecting resistance to post-emergence ACCase and ALS inhibitor herbicides in grass weeds. Weed Research 51(3), 284–293 (2011).
11) Furlong, M. J. et al. Diamondback moth ecology and management: problems, progress and prospects. Annual Review of Entomology 58(1), 517–541 (2013).
12) Thrall, P. H. et al. Evolution in agriculture: the application of evolutionary approaches to the management of biotic interactions in agro-ecosystems. Evolutionary Applications 4(2), 200–215 (2011).